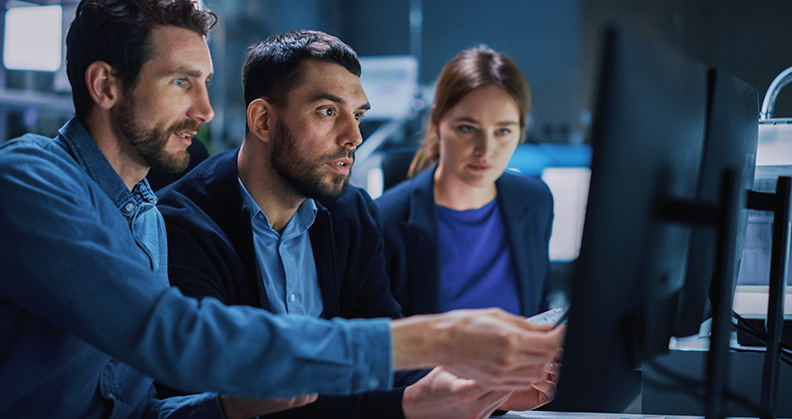
At a basic level, the computational design of orthopedic implants involves the use of computer algorithms, simulations and data analysis to enhance how devices ultimately function. Matthew Shomper, Principal Engineer of the consulting firm Not a Robot Engineering, believes advanced computational techniques are key to allowing product development professionals to enhance the form and function of additively manufactured implants.
“Most of the workflows that I build for customers include some sort of variables for which they can adjust parameters, but the way computational design goes about those steps is a little bit more intensive,” Shomper said. “It might include simulations or other calculations outside of what the engineer can directly control through data input.”
According to Shomper, the need for computational design arises from current understandings of complex biological principles like Wolff’s Law and Frost’s mechanostat theory. He said Wolff’s Law suggests that bone adapts to its local environment through mechanical loading. Essentially, bone grows when subjected to a certain amount of load and resorbs when that load is absent.
Frost’s mechanostat theory further elaborates on this concept, proposing that there’s a mechanism by which stress and strain in bone are monitored. According to this theory, bone remodels itself in response to specific types of overloads, adding or removing tissue as needed.
“Certain rates of strain are more beneficial for bone health,” Shomper said. “Given this complexity, computational design becomes crucial. It allows us to leverage algorithms, simulations and data analysis to predict and enhance how implants and devices interact with the body.”
Addressing Current Limitations
Computational design helps engineers model how mechanical loads affect bone growth and remodeling, allowing them to create more effective and personalized devices that work harmoniously with the body’s natural processes. Shomper said this level of precision and adaptability could not be achieved with traditional parametric design.
“When we design implants, they serve as surrogates that are intended to replace a portion of the body,” he said. “However, from a biological standpoint, these implants often perform poorly compared to the original tissue they replace. Unlike bone, which can adapt and remodel in response to mechanical loads, implants generally lack this dynamic ability.”
The idea of an overload zone, where bone begins to remodel itself under certain conditions, is well-known, Shomper noted. “Yet, when we design an implant to replace bone and use materials that aren’t biologically similar, we often encounter a stiffness mismatch,” he said. “This mismatch can lead to complications because the implant doesn’t behave like the natural bone it replaces.”
Shomper said that bone, particularly trabecular bone, can resorb and remodel itself. “This adaptive capability is something current implant design technology, even with computationally designed products, can’t fully replicate,” he said. “We therefore need to be cautious when designing these surrogates. Just because a device looks like trabecular bone doesn’t mean it’s functionally equivalent, especially if it’s made of titanium. The key is to measure the actual force output and impact on the body.”
Shomper noted the importance of understanding what happens to the body at macro, micro and nano levels. For example, he said, cyclic loading of bone at less than 1% of its ultimate strength can cause measurable changes in microstrain and its ability to trigger remodeling.
“When we use materials with a modulus mismatch — whether it’s PEEK, titanium or an allograft — we’re dealing with a potential problem. Any mismatch in the material’s properties compared to native bone can have significant implications,” Shomper said. “Bone is not a static material. Its modulus changes and adapts in response to the environment. Designing implants that fail to account for these dynamic properties could lead to suboptimal outcomes and limit the effectiveness of the treatment.”
As computational design advances, engineers can aim to lower the stiffness of implants to better match the modulus of bone. However, Shomper said this adjustment alone might not be sufficient without changes in how 3D-printed products are regulated. He pointed out that although some engineers have created low-stiffness devices, they often face limitations related to strength and durability in cyclical testing.
Shomper used the term “biological footprint” to describe an implant that is ideally integrated and effectively forgotten by the body. He believes the best implants seamlessly integrate into the natural anatomy and function without causing additional issues or requiring ongoing attention.
“Looking ahead, we may move toward biologic additives that can resorb into the body and leave only natural bone behind,” he said. “While we’re not quite there yet, an ideal scenario would involve maintaining stiffness in implants while enhancing strength. This remains a challenging problem to solve.”
Embrace the Future of Implant Design
No two anatomies are identical. Geometry, loading conditions and body sizes and shapes vary among individuals. “Even with parameterized design — where we carefully adjust parameters such as height, width and lordosis — we still can’t achieve a perfect anatomical match,” Shomper said. “This is where computational design becomes valuable.”
Shomper noted that computational modeling enables the printing of ordered and random structures that can reduce stiffness and adapt to various loading conditions. He said grading 3D-printed structures based on density and morphology can better accommodate individual anatomical differences.
Finite Element Analysis (FEA) plays a crucial role in this process by allowing engineers to model structures digitally and use the results as a feedback loop to inform and refine implant designs. This iterative process can be applied during the development phase or as part of patient-specific solutions. Computational modeling also facilitates the analysis of massive data sets, further enhancing the ability to design implants that meet individual patient needs and optimize performance.
“We can create more accurate bone surrogates compared to many existing designs,” Shomper said. “These advanced structures allow for greater customization and optimization, making them more effective at mimicking the properties of natural bone.”
Making functional adjustments and grading the material of an implantable device — moving it from areas of low stress to areas of high stress — can significantly optimize the design by redistributing material. Engineers essentially add it where it’s needed and remove it from where it’s not.
“Even small shifts in material distribution can profoundly impact the stiffness and other properties of the structure,” Shomper said. “Computational modeling enables us to achieve these adjustments with a high degree of accuracy, allowing us to accomplish feats that were previously considered impossible.”
Computational modeling, when combined with FEA, allows engineers to better understand and address adaptive stresses, leading to more effective and optimized implant designs.
“It allows us to use iterative feedback to continuously improve our models,” Shomper said. “We build on the results from the first and second iterations. Analysis of the newest structure will determine if it performs optimally, with all parameters remaining within safe limits. If the orientation or loading conditions aren’t quite right, we can easily adjust them.”
One of the exciting aspects of computational modeling is its speed. Tasks such as meshing algorithms and performing simulations can now be completed in a fraction of the time. This rapid processing enhances the ability of engineers to iterate and refine product designs.
Shomper acknowledged that the learning curve for many advanced design software tools can be steep, especially when they’re used to create cutting-edge implant designs. He recommends that engineers start with a solid understanding of lattice structures and their applications and how to use them effectively.
“The required domain knowledge is extensive, particularly when you incorporate biological aspects such as pore size, surface roughness and the interplay of micro and macro features,” Shomper said.
Still, Shomper said device engineers shouldn’t hesitate to invest in modern design tools. He noted that advanced software can offer new capabilities to CAD platforms and believes many of these newer tools will complement traditional parametric systems rather than replace them, providing enhanced functionality and flexibility.
“Traditional tools remain valuable, but incorporating advanced design software into your product development portfolio is crucial as the field of lattice structures and metamaterials expands,” Shomper said. “Lattices and metamaterials are not just structural. They have diverse applications, including in biological fields, heat transfer, vibrations and optical technologies. Embracing these innovations can provide significant advancements in implant design.”
DC
Dan Cook is a Senior Editor at ORTHOWORLD. He develops content focused on important industry trends, top thought leaders and innovative technologies.